Our research aims to understand the events underlying early mammalian development. The shape of fertilized eggs of mammals is spherical, but after rounds of cell division, clear axes are established in embryos, and various differentiated cells are arranged in an orderly fashion along the body shape. By carefully observing the behavior of individual cells, we try to determine how the embryo is formed as a group of cells during development through communication between tissues and cells. Focusing on early mouse embryos, we study mammalian embryonic development by analyzing individual cells, protein functions, gene expression regulatory mechanisms, and the physical properties of cells and tissues. To address fundamental questions in developmental biology and to elucidate the mechanisms underlying embryonic axis formation, morphogenesis, and cell differentiation in mice, we use a variety of techniques, including advanced microscopy, genetic manipulation, developmental engineering techniques, and mathematical modeling. A major feature of mammalian embryonic development is that it occurs within the maternal organs such as the oviduct and uterus, and the interaction between the embryo and the maternal tissues is also important for embryonic development. During implantation, the morphology of the uterus changes in response to signals from the embryo, and at the same time, the state of the embryo changes dynamically, establishing a relationship between the mother and the embryo. The uterine tissues support embryonic development after implantation not only by providing nutrients, but also by the action of various molecules, and by mechanical forces exerted on the embryos. By studying the early mammalian embryo, which slowly progresses in the materialization of information, we aim to understand the high capabilities of living organisms.
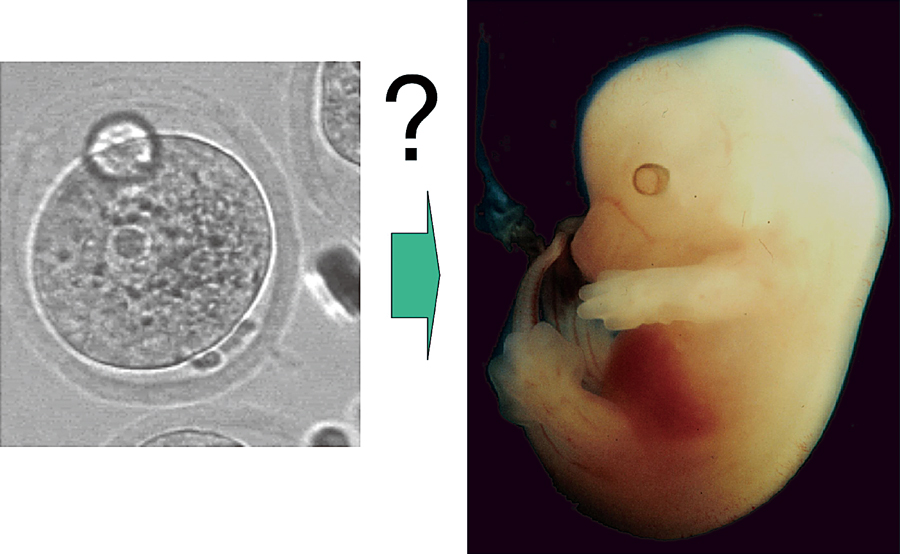
A mouse fertilized egg and an embryo 12 days after fertilization. An embryonic body with axes of anterior-posterior, dorsal-ventral, and left-right is formed from an egg with a symmetrical shape. How is the information relating future body formed during the early stages of development?
Research Projects
-
• Mechanisms of embryo implantation in mouse
-
• Interactions between the uterus and embryo during the formation of embryonic axes
-
• Control of morphogenesis by mechanical forces
-
• Formation and maintenance of planar polarity in epithelial tissues
I. Live observation of early mouse development
To understand the mechanisms underlying embryonic development and morphogenesis, it has become common to observe the behavior of cells and gene expression in living embryos in a variety of animal species. Progress in genetic engineering and embryonic manipulation concomitantly with the progress in optics and microscope technologies, including development of highly sensitive photo-detectors, has allowed us to observe developing embryos, even mammalian ones, in real time. We have established a series of transgenic mouse lines for live imaging, which is part of a collaborative project taking place in the Laboratory for Animal Resources and Genetic Engineering, Riken CLST. These mouse lines have been used to visualize nuclei, cell shapes, the cytoskeleton and other organelles to observe cell behaviors in living mouse embryos across many laboratories over the world. We have also established mouse lines to monitor the cell cycle. We have been studying the behavior of cells within embryos by applying newly developed image analyses following the observation of cell behaviors within embryos using these mouse lines. We found that the proximal visceral endoderm overlying the extra-embryonic ectoderm shows coherent cell growth in a proximal-anterior to distal-posterior direction. We also observed that directional cell migration is coupled with cell elongation in the anterior region, suggesting that the behaviors of visceral endoderm cells vary between regions during peri-implantation stages.
We have also been establishing several reporter mouse lines in the lab to study gene expression patterns during the peri-implantation stage of mouse development. In these mice, cDNA encoding fluorescent proteins are connected to the mouse genomic DNA sequences of the enhancer/promoter region of important genes encoding factors regulating cell differentiation in these stages.
II. Histological observation of mouse embryos developing in the uterus
Mammalian embryos develop in the uterus after implantation. Direct interaction between embryos and the cells of the uterus is important. In studies on the developmental biology of mammalian embryos, embryos are usually removed from the uterus, and those that are isolated are analyzed. We have been analyzing the early embryonic development of the mouse by comparing the changes in the uterine epithelium. To observe the morphogenesis of embryos within the uterus, serial sections of implanted uteruses were made, and images of the embryos within it were captured to make high resolution three-dimensional re-constructions. We are preparing samples obtained from various developmental stages. Precise positions of embryonic implantation and changes in the uterine epithelium correlating with embryonic development have been examined using these images. We are identifying the molecules involved in the interaction between the embryo proper and uterine cells, which may play a major role in embryonic development.
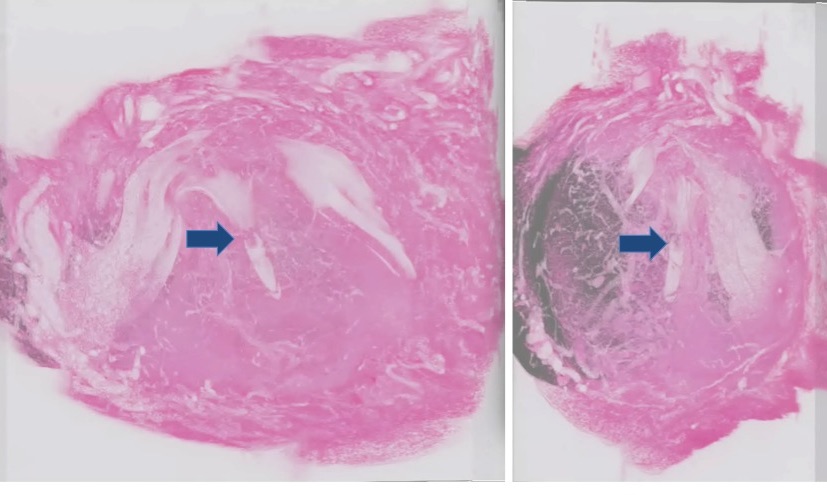
Figure 1. Three-dimensional image reconstruction of the pregnant uterus. A uterus obtained 5 days after fertilization was sectioned and images were digitized by an image scanner, and reconstructed three-dimensionally to observe the relationship between the embryo and the maternal tissue. The right panel represents the pseudo 3D image observed from the lateral side of the left image. Arrows indicate the embryo in the decidual tissue.
III. Polarity formation in the mouse oviduct epithelium
The oviduct (Fallopian tube) is the organ connecting the periovarian space and the uterine horn. The ova released from the ovary are transported through the oviduct, where the ova are fertilized by spermatozoon entering from the uterus. The epithelium of the mouse oviduct consists of multi-ciliated cells and secretory cells. Ovulated oocytes are transported in oviducts by unidirectional motility of multi-cilia. This cellular orientation in the oviduct is one example of planar cell polarity (PCP). PCP is seen in a variety of tissues, where cells sense global axes of the tissue to which they belong and orient themselves to fulfill specialized functions.
Many evolutionally conserved genes are required for the formation of PCP, and several of those encode proteins that are localized in a polarized manner within cells. We found that Celsr1, a PCP core member, was strongly concentrated only at the specific domains of cell boundaries which are perpendicular to the ovary-uterus axis, and that this polarized localization appeared to precede the directional movement of cilia. In
Celsr1-deficient mutant oviducts, the beating direction of cilia was not coordinated along the ovary-uterus axis throughout the epithelia; some cells were oriented orthogonally to the axis, while some others exhibited reverse polarity. Recently, we found that Camsap3, a microtubule minus-end binding protein, localized to the uterine side of the base of cilia and its mutation disrupted cilia orientation in individual cells without affecting Celsr1 localization. Thus, both Celsr1 and Camsap3 are responsible for the alignment of cilia along the ovary-uterus axis, but in distinct ways; Celsr1 orients cells, while Camsap3 orients cilia within each cell.
In addition to the ciliary polarity phenotype, cellular shape polarity was also impaired in the
Celsr1-deficient mice. We found a new feature of cellular polarity in the wild type oviduct,
e.g. that the apical surface of epithelial cells was elongated along the ovary-uterus axis. In
Celsr1-deficient mice, epithelial cells showed less elongation and a randomized orientation.
Furthermore, we also found that the three-dimensional architecture of oviductal epithelia was dramatically disrupted. In adult wild-type mice, the epithelial cell sheet is bent multiple times, which forms around twenty longitudinal folds parallel to the organ axis. However, in the mutant oviducts, epithelial folds no longer run parallel to the organ axis. The direction of the folds was randomized, and ectopic branches of the folds were observed. This suggests that Celsr1 is important for the formation of multi-scale polarities in the mouse oviduct ranging from the cellular to the tissue scale.
Epithelial folds are observed in various organs including the oviducts, guts, and airways etc. Longitudinally aligned folds are also observed in the oviducts of birds and frogs. To investigate the mechanisms of the epithelial fold pattern formation, we utilized mathematical modeling and simulations. By considering mechanical properties of the epithelial sheets, we reproduced the longitudinally aligned and branched folds which are observed in wild-type and
Celsr1 mutant mice, respectively (Figure 2). In addition, our model also successfully reproduced circumferentially aligned folds and zigzag folds observed in other organs. We are also focusing on some other PCP regulators, and are trying to understand how oviduct epithelial cells establish and maintain their polarity.
Figure 2. Epithelial fold patterns in oviduct and the reproduction of the patterns by computational simulations.
IV. Analysis of the mechanical properties of cells and tissues during embryonic development
Mechanics is one of the essential components of biological processes, including cell shape transformation and tissue morphogenesis etc. To understand how mechanical forces contribute to various patterns of morphogenesis, measuring cellular and tissue mechanical states is necessary. We developed statistical techniques to infer mechanical states using fluorescent microscopic images during morphogenesis (Figure 3). By employing this method, we inferred mechanical forces in multi-cellular systems including cultured epithelial cells, and early embryogenesis in
C. elegans and mice. Further computational simulations based on the inferred mechanical information reproduced morphological features of the multi-cellular systems. Thus, the mechanical information will be useful to investigate physical mechanisms of early embryonic development and morphogenesis during organogenesis in late stages of development.
Figure 3. Theoretical inference of cellular/tissue mechanical states. Schematic illustration of inference.
V. Mammalian tissue morphogenesis requiring mechanosensor channel PIEZOs
Several examples have shown that mechanical stimuli can work as key components for tissue or organ development. However, our knowledge about the involvement of mechanotransduction in biological phenomena or their precise mechanisms is still limited. It is partially because key mechanosensors are not yet identified in many cell types. PIEZOs are recently identified mechanically activated cation channels functioning in mammalian cells (Figure 4). They are activated when mechanical forces are applied to the cell membrane. Series of data show that PIEZO2 serves as the main mechanosensor in sensory neurons for light touch sensation, proprioception and breathing. We recently found that PIEZO1 in endothelial cells is required for lymphatic valve formation (Figure 5). To further elucidate how PIEZO1-mediated mechanotransduction is involved in lymphatic valve formation, we have been developing systems to manipulate mechanical stimuli and monitor PIEZO activity in vitro and in vivo. Analyses utilizing these systems and mouse lines deficient in PIEZOs will clarify the relationship among mechanical forces, PIEZO activation, cellular responses, and tissue morphogenesis.
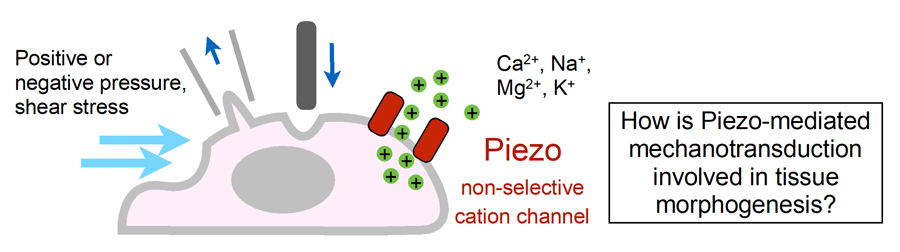
Figure 4. Schematic illustration of Piezo mechanically activated non-selective cation channel and the key question of this study.
Figure 5. Reduced number of lymphatic valves in mice lacking PIEZO1 in endothelial cells.
VI. Mechanics of cell population patterning during development
During development, cells actively and/or passively move, resulting in various cell distribution patterns. We investigated the effect of passive cell movements provoked by frictional forces from adjacent growing tissues. The passive movements generated various patterns, such as an elongated cell cluster and multiple cell clusters, etc. Difference in cellular stiffness was critical for these pattern formations. The former situation is actually observed during elongation of the notochord in mice. Together with active cell movements and the differential adhesion hypothesis which has been recognized as a classical concept within biology, passive cell movements can be effective for diverse pattern formation.